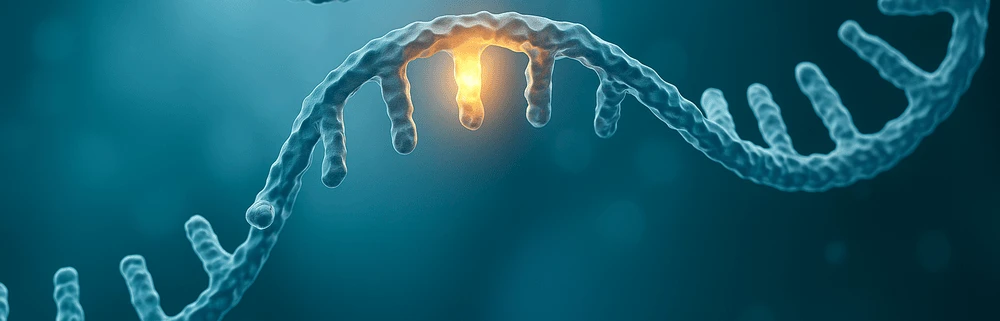
The Hidden Code of RNA
In the flow of genetic information, messenger RNA is the gateway between the genetic code and cellular function. Though its core function in protein synthesis has been studied extensively, scientists are only now beginning to explore the array of chemical modifications that fine-tune its function, stability, and regulation. These modifications, referred to as the “epitranscriptome”, provide a dynamic layer of gene expression control, influencing everything from development to disease progression.
Why RNA Modifications Matter
Gene expression regulation is an essential, multifaceted feature of all forms of life. It helps explain the vast diversity in cell types, functions, and responses to external stimuli, despite sharing the same DNA sequence. For example, the human body comprises more than 200 distinct types of cells, with protein expression levels in each ranging from 10s of copies to millions of copies, as required by that cell type, current condition, and protein function. Epigenetic and epitranscriptomic mechanisms are key regulators that govern which genes are expressed, when, and at which level, bridging the gap between genotype and phenotype.
Types of RNA Modifications
Because life sets high demands for the regulation of gene expression, multiple mechanisms exist to modulate both DNA and RNA. Key players at the DNA level include gene methylation and histone modifications, which are slower acting. At the RNA level, multiple types of chemical modifications occur that diversify the structure beyond the ribose–phosphate backbone and the A, C, U, and G nucleobases. Some of these modifications are well known in the structure and function of tRNA, but many more play regulatory roles in seemingly all aspects of RNA biology, profoundly influencing secondary and tertiary structure, stability, protein–RNA interactions, alternative splicing, transcript lifetime and trafficking, translation rate and errors, and stress granule formation.1 These modifications can be installed co-transcriptionally to tune RNA function as it is formed or can be added and removed dynamically to change RNA properties during the lifetime of the transcript.
How RNA Modifications Work
The most abundant and best-studied RNA modifications found in mRNA involve chemical changes to the nucleobases. These modifications are small changes with respect to the canonical structures of A, C, U, and G, typically no larger than the addition of a methyl or hydroxymethyl group. Among these, N6-methyladenosine (m6A) is the most abundant internal modification in eukaryotic mRNA, occurring at approximately 3 to 5 sites per transcript and accounting for 0.1% to 0.4% of all adenosines. Though the addition of a single methyl group is a subtle change to the amino group of adenosine, it has profound structural implications. m6A naturally adopts a conformation with the methyl group facing the Watson–Crick edge, disfavoring base pairing, although the C–N bond can rotate to position the methyl group on the Hoogsteen edge to enable canonical hydrogen bonding. Accordingly, the methyl group of m6A is structurally significant because it alters the stability of base pairing and changes the pattern of hydrogen bond donors and acceptors at either the Watson–Crick or Hoogsteen edge. Other common base modifications, such as m1A and m7G, similarly influence RNA structure and function through changes to hydrogen bonding or base pairing. Pseudouridine (Ψ), which is isosteric to uridine and found at similar frequencies (3–5 sites per transcript), adds a hydrogen bond donor to the major groove edge. Inosine (I; 1 – 3 sites per transcript), which is formed from adenosine through the action of a deaminase enzyme (ADAR), has a hydrogen bonding pattern similar to guanine, but without guanine’s N2 H-bond donor. The rarer modification, 5-methylcytosine (m5C; 0.3 – 1 sites per transcript), is unique in that it adds a methyl group to the already hydrophobic C5 of cytosine, which does not alter the hydrogen bonding properties of the nucleobase.
Because most RNA modifications change the hydrogen bonding character of the nucleobases, they are able to directly exert regulatory and structural functions, even without reader proteins. However, RNA-binding reader proteins remain critical mediators of modification-dependent functions, adding yet another layer of dynamic control to this regulatory system. Broadly, proteins that interact with RNA modifications fall into three functional classes: writers, readers, and erasers, aptly named for their roles in installing, interpreting, and removing these chemical marks. All RNA modifications are associated with writer enzymes that install the modifications either co- or post-transcriptionally. Among the best studied enzymes are the METTL3–METTL14 enzyme complex that installs m6A on mammalian RNAs in the nucleus, including nascent mRNA. Recent work shows that the installation of m6A is tightly linked to the splicing process.2 Inosine is installed by deaminases that act on adenosine in RNA (ADAR enzymes) and pseudouridine is installed by pseudouridine synthases (PUS enzymes). Because RNA is turned over much more rapidly than DNA, not all RNA modifications require eraser enzymes. m6A can be removed from mRNA by FTO (fat mass and obesity-associated protein), but inosine and Ψ do not have known eraser enzymes.
RNA Modifications in Health and Biotechnology
It is clear that RNA modifications are essential regulators of gene expression and RNA function. CRISPR-based knockdown of enzymes in RNA-modifying pathways severely impacts cell viability and knockout can prevent cell growth, proving that these are vital pathways. But because the effects of RNA modifications depend on the gene locus in which they are found, there is no one general effect that can be ascribed to each modification type for a given transcript of interest. Accordingly, we highlight some recent examples of RNA modification function in the biology of human disease and in biotechnology.
One exciting area of research on RNA modifications is in cancer biology. Despite observations that RNA modification function can be locus-specific, it has been found that the global presence of m6A is associated with pathways of cancer cell proliferation. In one seminal study by Gregory et al., the authors find that shRNA knockdown of the m6A writer METTL3 depletes m6A levels from multiple oncogene transcripts, leading to less translation and reduced cancer cell growth and proliferation.3 In related work, Kouzarides et al. of STORM Therapeutics have developed STC-15, a small molecule inhibitor of METTL3 that, through suppression of adenosine methylation, slows the growth of acute myeloid leukemia cells and prolongs survival in a mouse model; this investigational new drug has recently completed a Phase I clinical trial. Multiple additional published studies present data showing significant aberration in the expression of m6A writer, reader, and eraser proteins in many cancer types and high levels of dysregulation of these proteins is associated with a poor prognosis.4 Accordingly, there is great opportunity for the further development of m6A as a biomarker for the early detection and diagnostics of cancer, for monitoring disease progression and for the success of therapeutics (see, for example, Figure 2).5–8
Similar potential exists for other key RNA modifications, including inosine and pseudouridine.9,10 One of the most significant recent findings on inosine and cancer is that there is a key role of ADAR1, an enzyme that converts adenosine to inosine, in the immune checkpoint blockade, the mechanism by which cancer cells resist being attacked by CD8+ T cells.11 The loss of ADAR1 profoundly sensitizes tumors to immunotherapy and overcomes resistance to the checkpoint blockade. Pseudouridine is less studied in cancer, but a striking example of its significance in seen in a 2024 study showing that the pseudouridine synthase PUS7 is essential for the growth and survival of MYC-driven cancer cells.12 MYC up-regulates PUS7 expression, which leads to increased pseudouridylation of the ATF4 mRNA. ATF4 is a master regulator of stress responses and cellular metabolism and is vital to MYC-driven cancer cell proliferation.
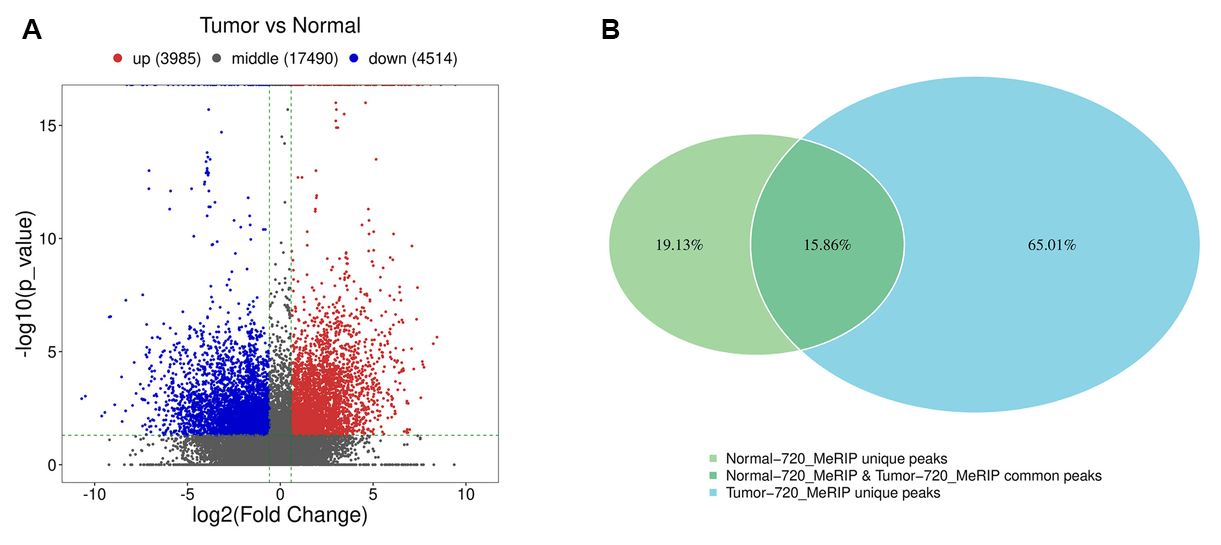
Figure 2: Abnormal levels of m6A modified mRNA are related to multiple pathways in breast cancer. A. The volcano plot of differential methylation sites of breast cancer tissues compared with adjacent normal tissues. B. Venn diagram (right panel) of common (intersection region) and unique peaks (non-intersection region) of breast cancer tissue compared with adjacent normal tissue. Modified from [13], licensed under CC BY 4.0.
An Accessible Epitranscriptome
RNA modifications are an exciting frontier in biological research. The selected examples discussed above only scratch the surface of opportunity to use RNA modifications as biomarkers for human health, to exploit RNA modification pathways in therapeutics and agriculture, and to advance basic research on gene regulation. With recent innovations, researchers now have improved tools to decode and harness the power of the epitranscriptome, unlocking new possibilities for science and human health.
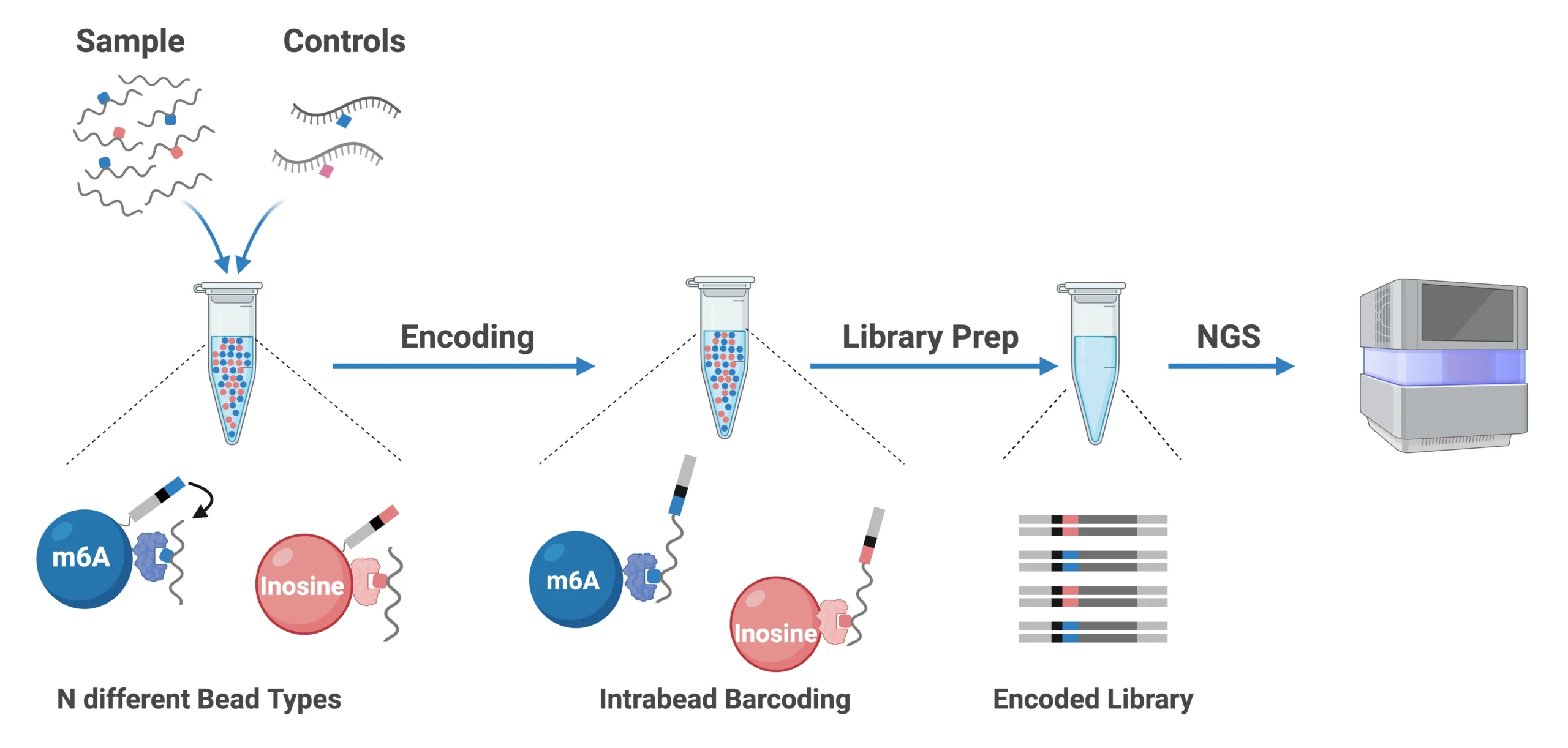
Figure 3: The EpiPlex Assay workflow enables users to generate sequencing-ready libraries from RNA samples in a single day. The current version supports multiplexed detection of m⁶A and inosine, with additional RNA modifications planned for future releases.
To accelerate the realization of these benefits, AlidaBio has developed the EpiPlex™ platform, which makes reading and quantifying changes in RNA modifications accessible to anyone doing RNA-Seq. The EpiPlex Platform offers a complete, antibody-free solution for RNA Modification detection and features:
-
- Simultaneous detection of m6A and inosine from as little as 20 ng of mRNA
- Proximity barcoding-based chemistry with synthetic spike-in controls for high accuracy
- Companion EpiScout bioinformatics pipeline for high-quality detection and quantification
- Standard readout on gene expression information through included RNA-seq
Combined with a streamlined, 1-day workflow and sequencing requirements as low as 25M reads, the EpiPlex Platform makes RNA modification studies highly accessible to all researchers.
About the Author
Byron Purse
Byron Purse is the co-founder of AlidaBio, a professor of chemistry at San Diego State University, and SDSU’s Director of the SDSU–UCSD Joint Doctoral Program in Chemistry. He earned a PhD in physical organic chemistry with Prof. Julius Rebek at Scripps Research, and his current research interests are focused on synthetic and natural modifications of nucleic acids. In addition to his work on technology and strategy at AlidaBio, Prof. Purse leads research at SDSU on synthetic fluorescent modifications of nucleic acids for live-cell RNA imaging and single-molecule fluorescence studies.
References
1. Roundtree, I. A.; Evans, M. E.; Pan, T.; He, C. Dynamic RNA Modifications in Gene Expression Regulation. Cell 2017, 169 (7), 1187–1200. https://doi.org/10.1016/j.cell.2017.05.045.
2. Yang, X.; Triboulet, R.; Liu, Q.; Sendinc, E.; Gregory, R. I. Exon Junction Complex Shapes the m6A Epitranscriptome. Nat. Commun. 2022, 13 (1), 7904. https://doi.org/10.1038/s41467-022-35643-1.
3. Lin, S.; Choe, J.; Du, P.; Triboulet, R.; Gregory, R. I. The m6A Methyltransferase METTL3 Promotes Translation in Human Cancer Cells. Mol. Cell 2016, 62 (3), 335–345. https://doi.org/10.1016/j.molcel.2016.03.021.
4.Deng, X.; Qing, Y.; Horne, D.; Huang, H.; Chen, J. The Roles and Implications of RNA m6A Modification in Cancer. Nat. Rev. Clin. Oncol. 2023, 20 (8), 507–526. https://doi.org/10.1038/s41571-023-00774-x.
5. Zhang, B.; Wu, Q.; Li, B.; Wang, D.; Wang, L.; Zhou, Y. L. m6A Regulator-Mediated Methylation Modification Patterns and Tumor Microenvironment Infiltration Characterization in Gastric Cancer. Mol. Cancer 2020, 19 (1), 53. https://doi.org/10.1186/s12943-020-01170-0.
6. Xu, X.; Zhu, H.; Hugh-White, R.; Livingstone, J.; Eng, S.; Zeltser, N.; Wang, Y.; Pajdzik, K.; Chen, S.; Houlahan, K. E.; Luo, W.; Liu, S.; Xu, X.; Sheng, M.; Guo, W. Y.; Arbet, J.; Song, Y.; Wang, M.; Zeng, Y.; Wang, S.; Zhu, G.; Gao, T.; Chen, W.; Ci, X.; Xu, W.; Xu, K.; Orain, M.; Picard, V.; Hovington, H.; Bergeron, A.; Lacombe, L.; Têtu, B.; Fradet, Y.; Lupien, M.; Wei, G.-H.; Koritzinsky, M.; Bristow, R. G.; Fleshner, N. E.; Wu, X.; Shao, Y.; He, C.; Berlin, A.; van der Kwast, T.; Leong, H.; Boutros, P. C.; He, H. H. The Landscape of N6-Methyladenosine in Localized Primary Prostate Cancer. Nat. Genet. 2025, 1–15. https://doi.org/10.1038/s41588-025-02128-y.
7. Wang, H.; Zhao, X.; Lu, Z. m6A RNA Methylation Regulators Act as Potential Prognostic Biomarkers in Lung Adenocarcinoma. Front. Genet. 2021, 12. https://doi.org/10.3389/fgene.2021.622233.
8. Thombare, K.; Vaid, R.; Pucci, P.; Ihrmark Lundberg, K.; Ayyalusamy, R.; Baig, M. H.; Mendez, A.; Burgos-Panadero, R.; Höppner, S.; Bartenhagen, C.; Sjövall, D.; Rehan, A. A.; Dattatraya Nale, S.; Djos, A.; Martinsson, T.; Jaako, P.; Dong, J.-J.; Kogner, P.; Johnsen, J. I.; Fischer, M.; Turner, S. D.; Mondal, T. METTL3/MYCN Cooperation Drives Neural Crest Differentiation and Provides Therapeutic Vulnerability in Neuroblastoma. EMBO J. 2024, 43 (24), 6310–6335. https://doi.org/10.1038/s44318-024-00299-8.
9. Stockert, J. A.; Weil, R.; Yadav, K. K.; Kyprianou, N.; Tewari, A. K. Pseudouridine as a Novel Biomarker in Prostate Cancer. Urol. Oncol. Semin. Orig. Investig. 2021, 39 (1), 63–71. https://doi.org/10.1016/j.urolonc.2020.06.026.
10. Zhang, Y.; Li, L.; Mendoza, J. J.; Wang, D.; Yan, Q.; Shi, L.; Gong, Z.; Zeng, Z.; Chen, P.; Xiong, W. Advances in A-to-I RNA Editing in Cancer. Mol. Cancer 2024, 23 (1), 280. https://doi.org/10.1186/s12943-024-02194-6.
11. Ishizuka, J. J.; Manguso, R. T.; Cheruiyot, C. K.; Bi, K.; Panda, A.; Iracheta-Vellve, A.; Miller, B. C.; Du, P. P.; Yates, K. B.; Dubrot, J.; Buchumenski, I.; Comstock, D. E.; Brown, F. D.; Ayer, A.; Kohnle, I. C.; Pope, H. W.; Zimmer, M. D.; Sen, D. R.; Lane-Reticker, S. K.; Robitschek, E. J.; Griffin, G. K.; Collins, N. B.; Long, A. H.; Doench, J. G.; Kozono, D.; Levanon, E. Y.; Haining, W. N. Loss of ADAR1 in Tumours Overcomes Resistance to Immune Checkpoint Blockade. Nature 2019, 565 (7737), 43–48. https://doi.org/10.1038/s41586-018-0768-9.
12. Ding, J.; Bansal, M.; Cao, Y.; Ye, B.; Mao, R.; Gupta, A.; Sudarshan, S.; Ding, H.-F. MYC Drives mRNA Pseudouridylation to Mitigate Proliferation-Induced Cellular Stress during Cancer Development. Cancer Res. 2024, 84 (23), 4031–4048. https://doi.org/10.1158/0008-5472.CAN-24-1102.
13. Xu, Y.; Song, M.; Hong, Z.; Chen, W.; Zhang, Q.; Zhou, J.; Yang, C.; He, Z.; Yu, J.; Peng, X.; Zhu, Q.; Li, S.; Ji, K.; Liu, M.; Zuo, Q. The N6-Methyladenosine METTL3 Regulates Tumorigenesis and Glycolysis by Mediating m6A Methylation of the Tumor Suppressor LATS1 in Breast Cancer. J. Exp. Clin. Cancer Res. 2023, 42 (1), 10. https://doi.org/10.1186/s13046-022-02581-1.
14. Yu, Q.; Liu, S.; Yu, L.; Xiao, Y.; Zhang, S.; Wang, X.; Xu, Y.; Yu, H.; Li, Y.; Yang, J.; Tang, J.; Duan, H.-C.; Wei, L.-H.; Zhang, H.; Wei, J.; Tang, Q.; Wang, C.; Zhang, W.; Wang, Y.; Song, P.; Lu, Q.; Zhang, W.; Dong, S.; Song, B.; He, C.; Jia, G. RNA Demethylation Increases the Yield and Biomass of Rice and Potato Plants in Field Trials. Nat. Biotechnol. 2021, 39 (12), 1581–1588. https://doi.org/10.1038/s41587-021-00982-9.
15. Wang, L.; Song, C.; Wang, N.; Li, S.; Liu, Q.; Sun, Z.; Wang, K.; Yu, S.-C.; Yang, Q. NADP Modulates RNA m6A Methylation and Adipogenesis via Enhancing FTO Activity. Nat. Chem. Biol. 2020, 16 (12), 1394–1402. https://doi.org/10.1038/s41589-020-0601-2.